Dosimetric Comparison of Proton Versus Photon Stereotactic Radiosurgery for Treatment of Vestibular Schwannoma
Images
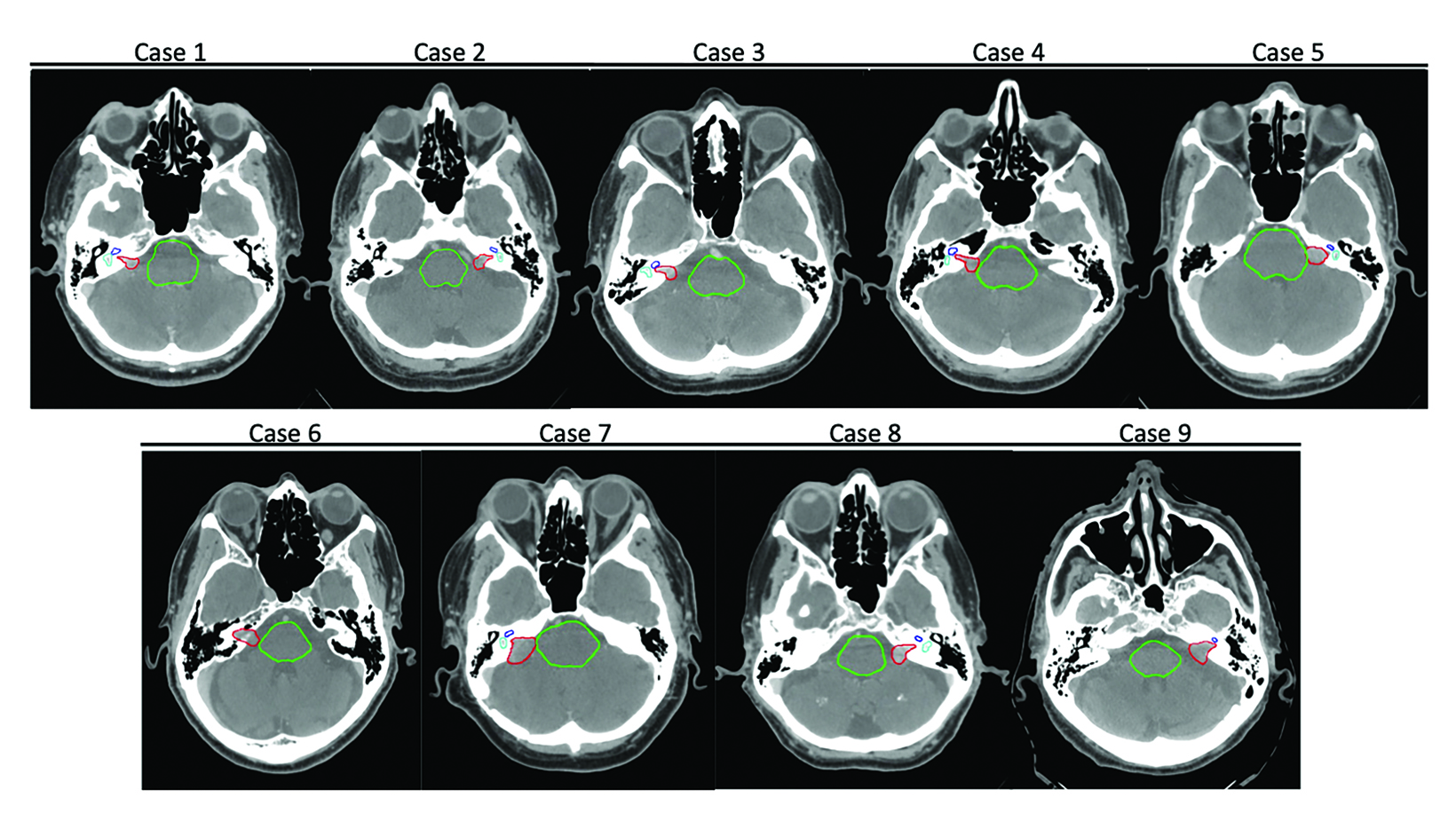
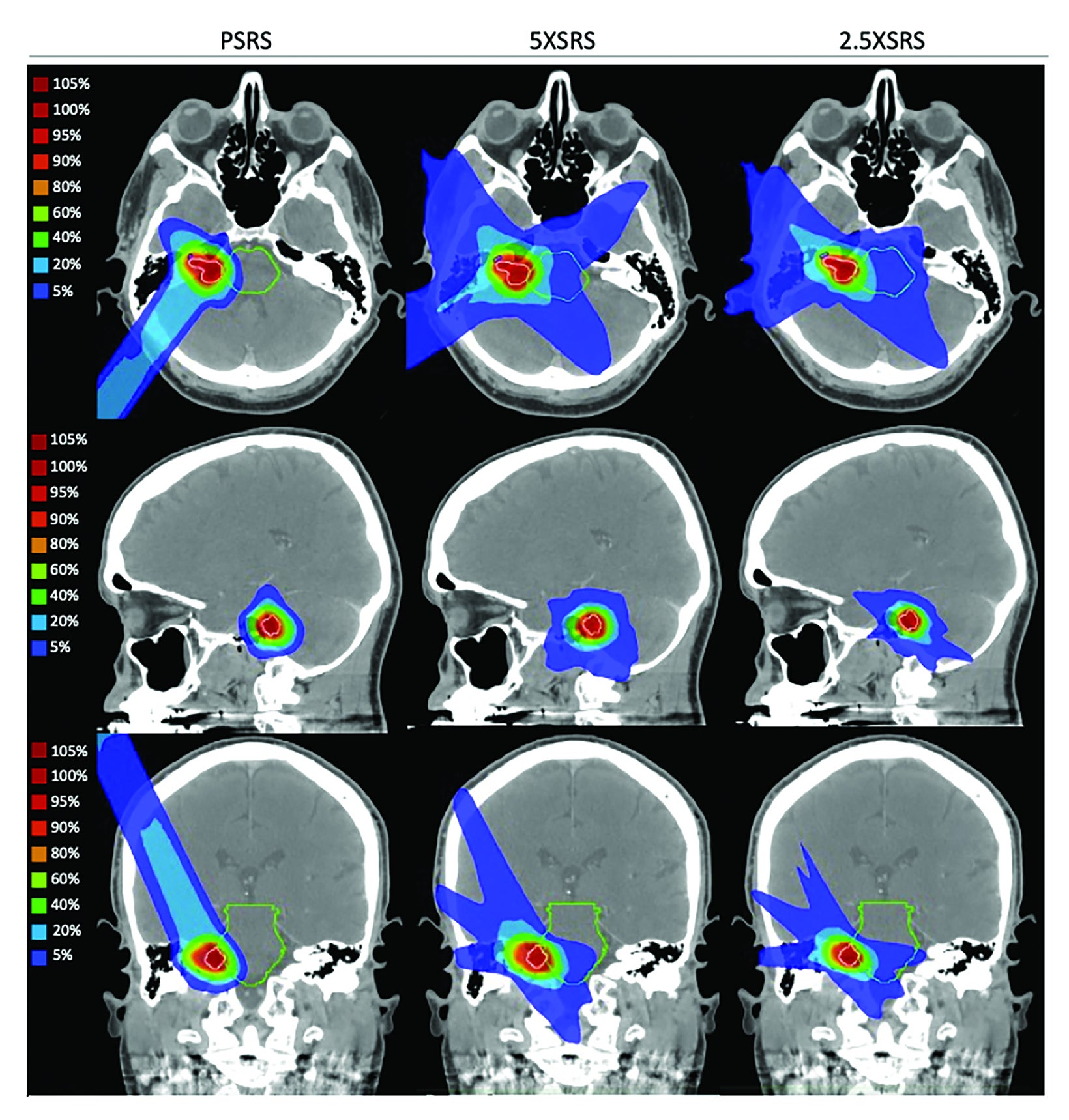
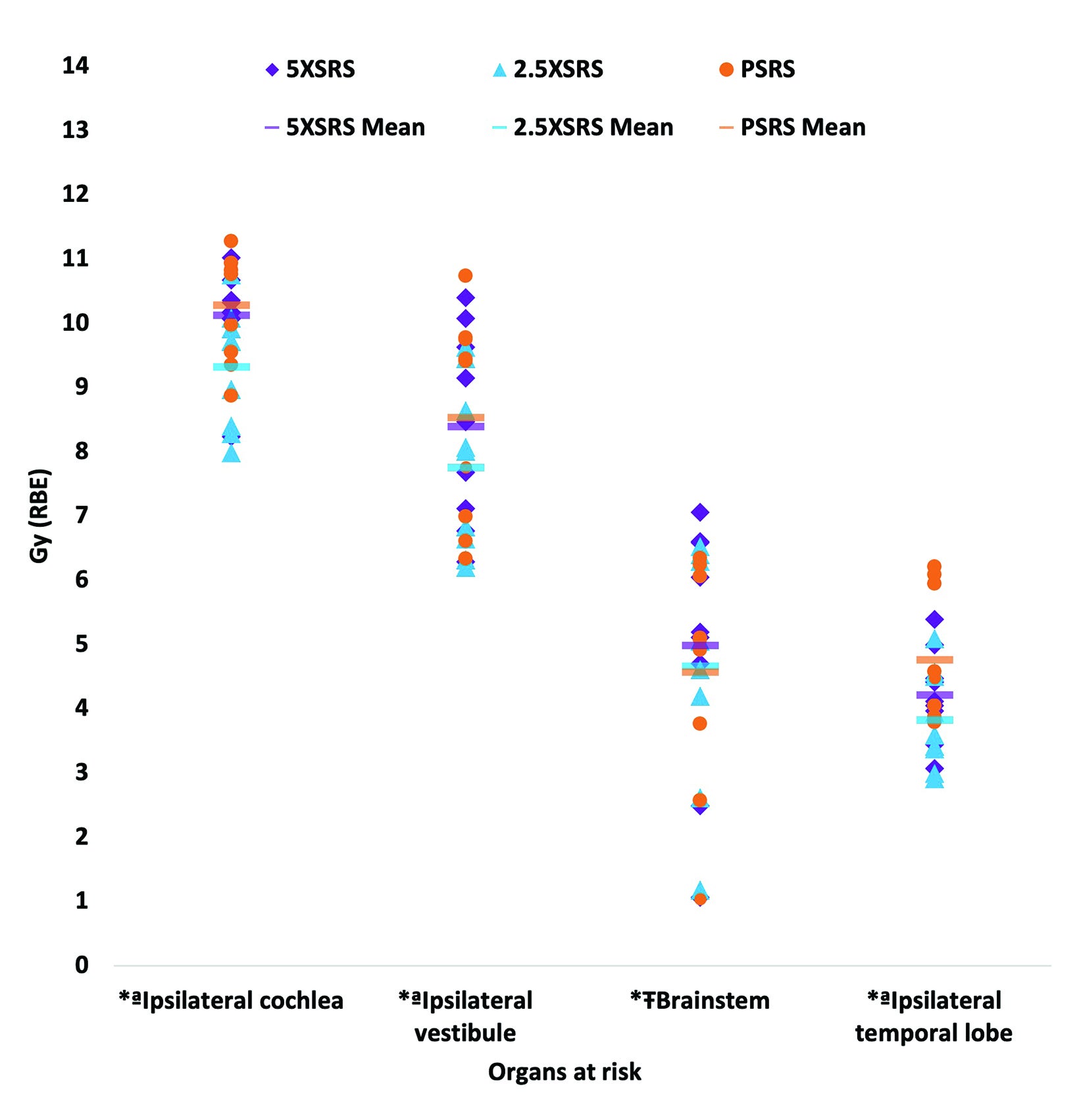
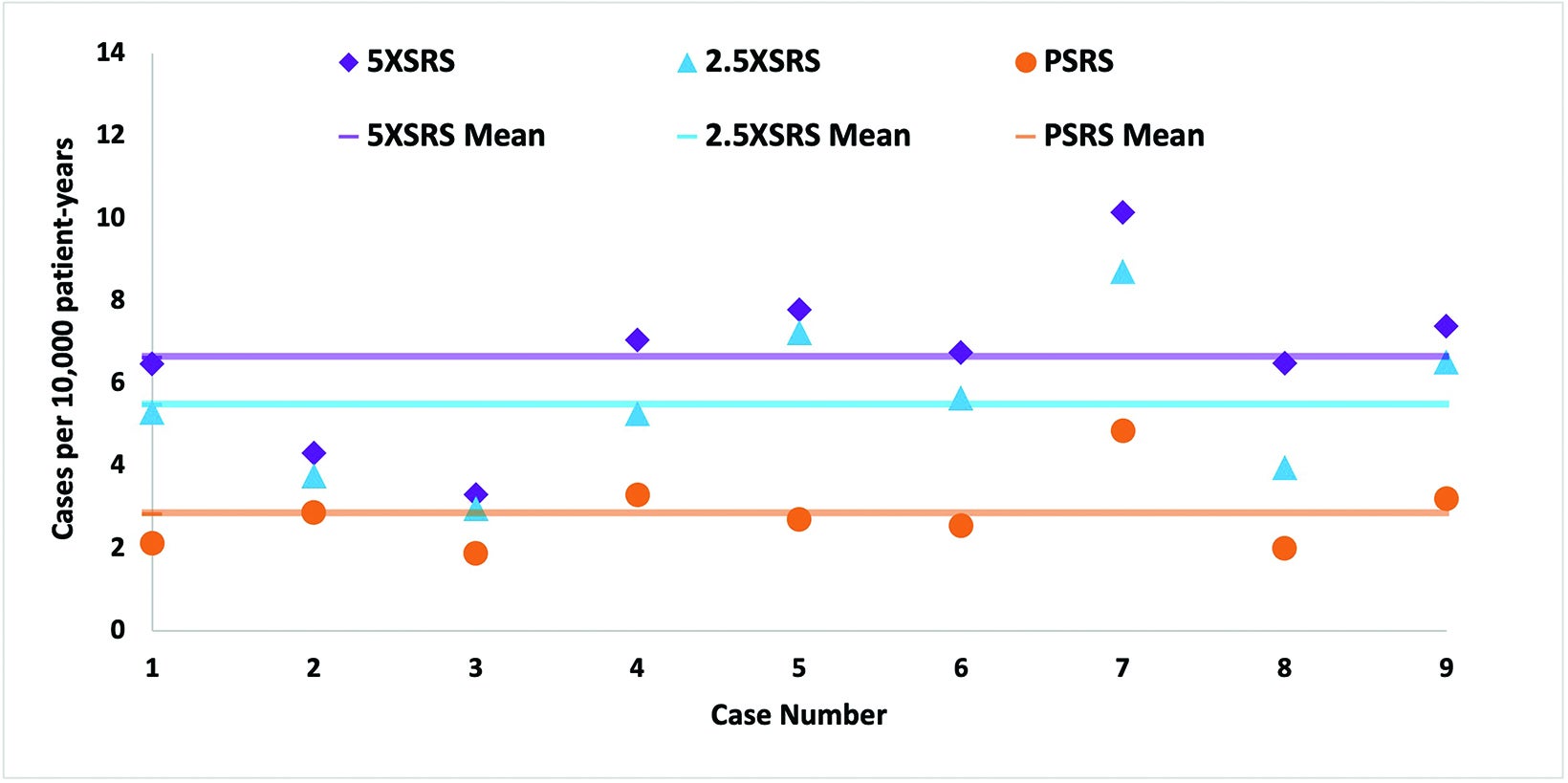
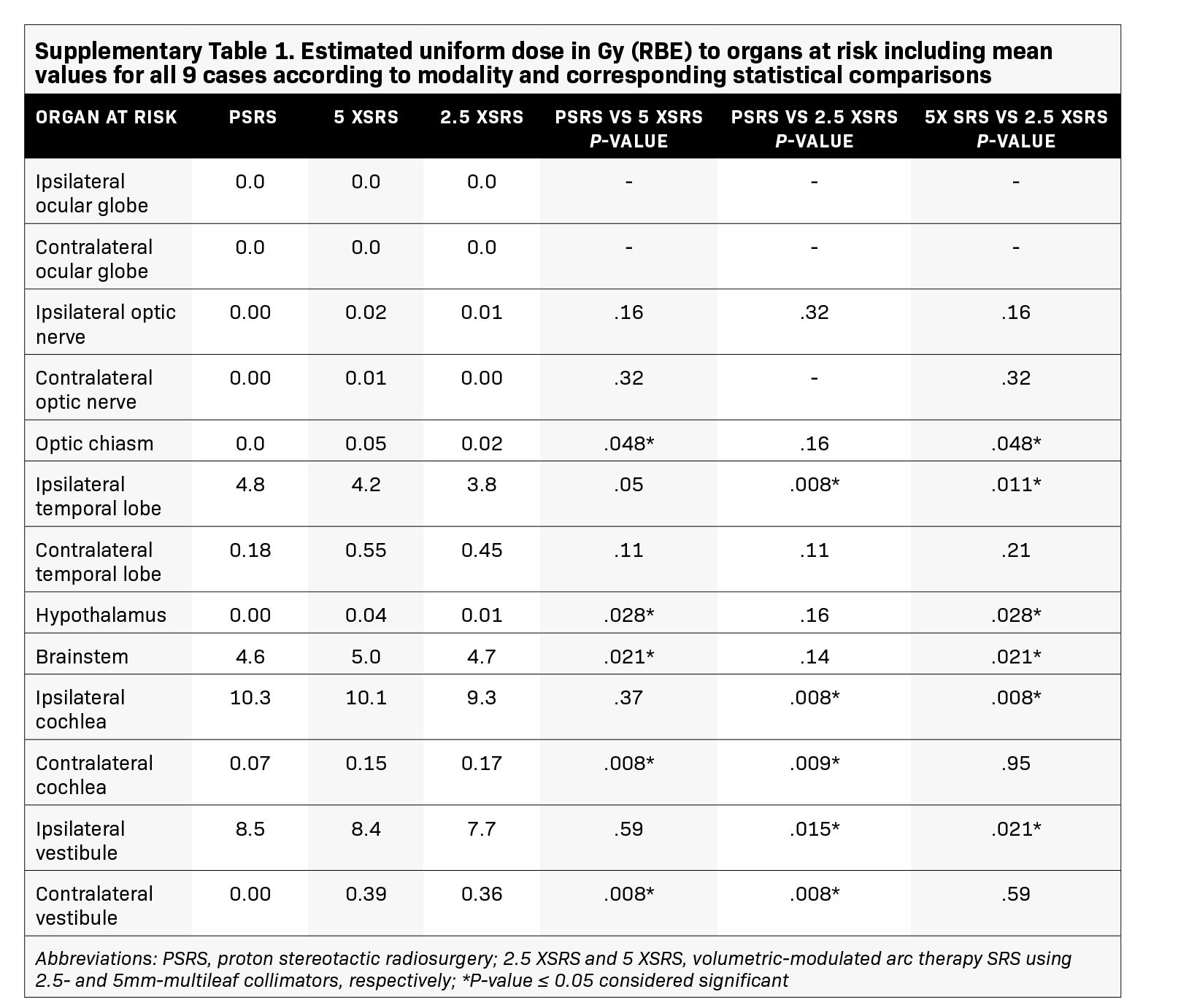
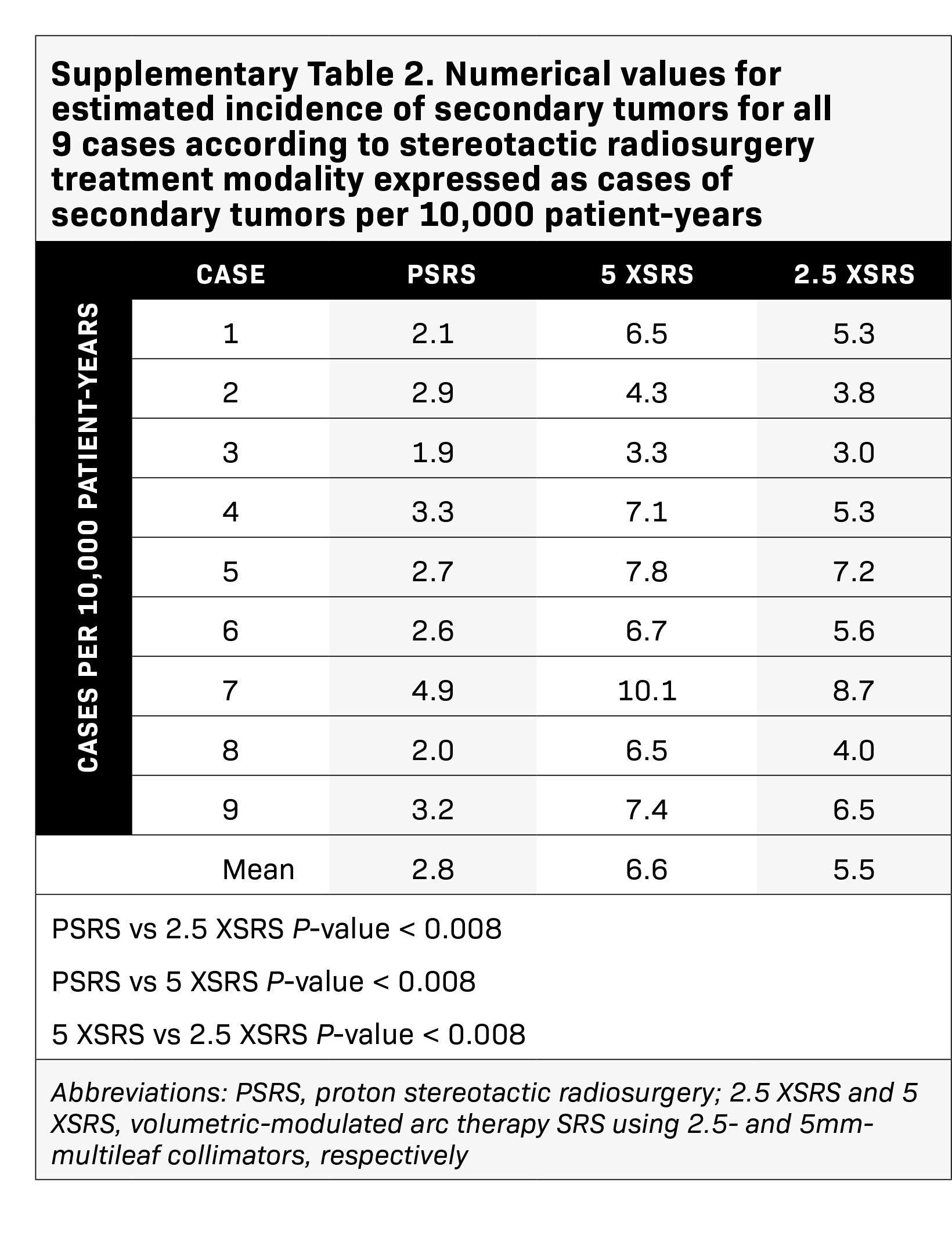
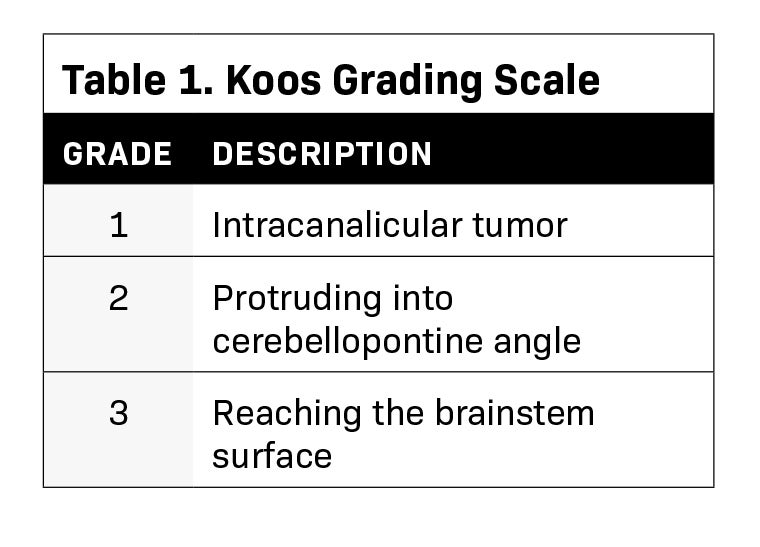
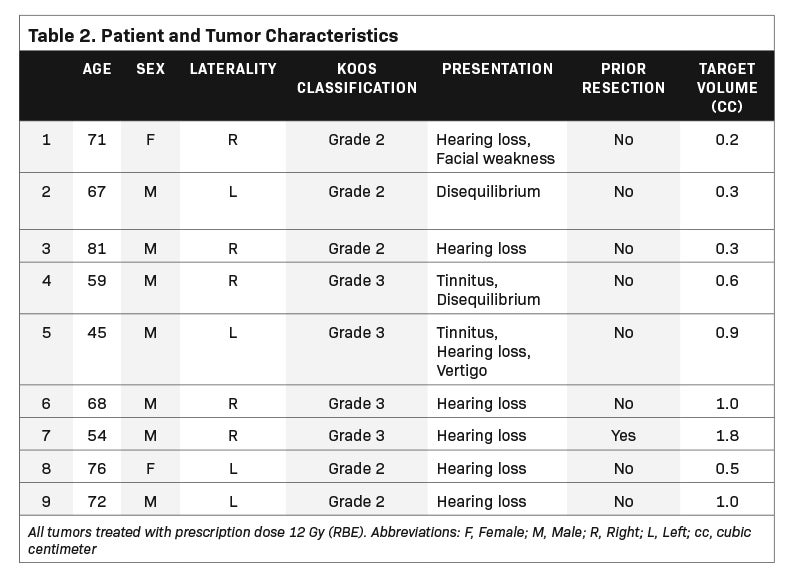
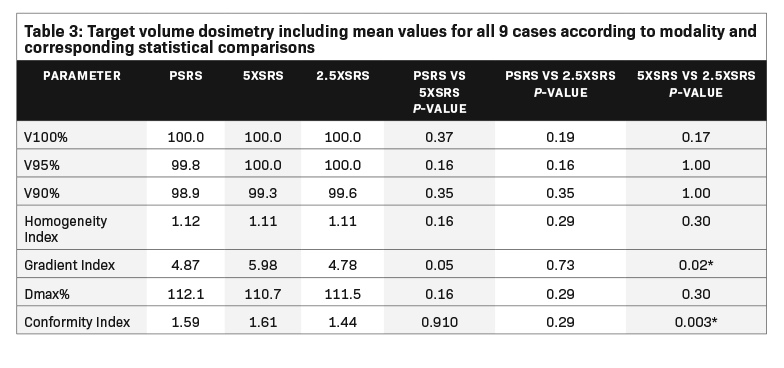
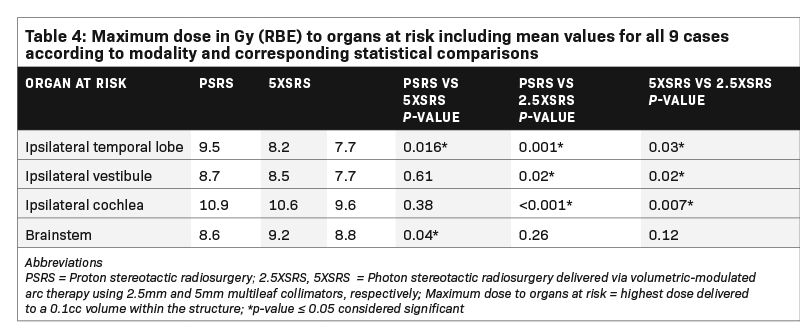
Abstract
Objective: To evaluate the dosimetric advantages and limitations of protons compared with photons in stereotactic radiosurgery for vestibular schwannoma.
Methods and Materials: Nine patients with vestibular schwannoma were selected among those receiving single-fraction proton stereotactic radiation therapy (PSRS) via a dedicated, passive, single-scattering stereotactic proton unit at a single institution between 2015 and 2018. These cases were re-planned with photon (X-ray) SRS (XSRS) volumetric-modulated arc therapy (VMAT) with 2.5- and 5-mm multileaf collimators (2.5 XSRS and 5 XSRS), respectively. Plans were constructed using the original total treatment dose of 12 Gy relative biological effectiveness (RBE) delivered in 1 fraction.
Results: Treatment plans were compared based on target volume dosimetry and estimated clinical toxicity. Average target volume was 0.71 cc (range, 0.2-1.8). There were no significant differences in V100%, homogeneity index or Dmax% between treatment modalities. However, 5 XSRS and 2.5 XSRS offered equal or superior V90% and V95% compared with PSRS for all 9 cases. Gradient and conformity indices were most optimal for 2.5 XSRS. Dmax in Gy (RBE) to ipsilateral temporal lobe (7.7, 9.5, 8.2), cochlea (9.6, 10.9, 10.6) and vestibule (7.7, 8.7, 8.5) was lower with 2.5 XSRS vs PSRS and 5 XSRS, P < 0.05. Dmax to brainstem was 8.8, 8.6, 9.2 for 2.5 XSRS, PSRS, 5 XSRS, respectively. Mean equivalent uniform dose (EUD) in Gy (RBE) to the ipsilateral temporal lobe, cochlea and vestibule was lower with 2.5 XSRS vs PSRS and 5 XSRS, P < 0.05. The projected risk of secondary tumors in excess of baseline was lowest for PSRS (PSRS - 2.8, 5 XSRS - 6.6, 2.5 XSRS - 5.5 cases per 10,000 patient-years; P < 0.008 for all comparisons).
Conclusions: This study compared the dosimetric advantages and limitations of PSRS, 5 XSRS and 2.5 XSRS for vestibular schwannoma. Target volume coverage and organ at risk (OAR) dose is similar between XSRS and PSRS; 2.5 XSRS offers greater target conformality and lower dose to OAR than 5 XSRS. PSRS offers significantly lower excess risk of secondary tumor than 2.5 XSRS and 5 XSRS although the absolute risk of secondary tumors is low across modalities.
Keywords: vestibular schwannoma, radiosurgery, proton therapy, acoustic neuroma
Vestibular schwannomas are benign cerebellopontine angle tumors arising from myelin sheath forming Schwann cells of the vestibular division of cranial nerve VIII. Common presentations include ipsilateral sensorineural hearing loss, dizziness, imbalance and asymmetric tinnitus.1 Large tumors may compress adjacent structures including cranial nerves (CN) V, VII, IX, X, XI, the brainstem, or cerebellum.
Overall incidence rates are estimated to be 3 to 5 cases per 100,000 person-years.2,3 Incidental diagnoses of vestibular schwannoma have increased in parallel with access to high-resolution imaging.1,2 Correspondingly, the treatment paradigm has evolved to include the options of conservative observation, microsurgery and radiation therapy (RT)4 based on tumor size, growth rate, hearing status, symptoms, patient age, comorbidities, and preferences.
RT is an effective treatment modality for vestibular schwannoma, especially for patients who decline surgery, are not surgical candidates due to comorbidity, or have surgically inaccessible or recurrent tumors. For appropriately selected cases, stereotactic radiosurgery (SRS) delivers high-dose conformal radiation to a limited target volume in a single fraction facilitated by high-precision localization in contrast to conventional regimens of 25 to 30 fractions.5 Both conventional fractionated external-beam RT and SRS achieve high rates of tumor control (84% to 100% at 5 years).5 Thus, treatment goals include minimizing long-term toxicities, including hearing loss, imbalance, and toxicity to other cranial nerves.
Feasibility of SRS depends on factors including tumor size and anatomical interface with the brainstem and cochlea if hearing is intact. SRS dose < 13 Gy are given to decrease risk of facial nerve dysfunction, trigeminal neuralgia and hearing loss.6,7 SRS may be delivered via several modalities including Gamma Knife (Elekta) or CyberKnife (Accuray), linear accelerators (linac), and proton units. Proton beams are highly conformal, have sharp lateral penumbras, low scatter, and preferential dose deposition within the target without exit dose due to its finite path length. As noted in The Congress of Neurological Surgeons evidence-based guidelines on vestibular schwannoma treatment, regarding radiosurgery technology, no studies directly compare SRS modalities, thus recommendations on outcomes based on modality cannot be made.4 Most studies of proton or photon SRS are single-institution experiences that do not directly compare these modalities but report similar tumor control efficacy.8,9 Furthermore, data are inadequate to compare hearing, cranial nerve preservation, cognitive function, and secondary tumors with each modality.8,9
In the absence of rigorous comparisons of SRS technologies, physicians must rely on theoretical benefits of each modality, limited series, and clinical experience. As protons are not widely available, patients may incur additional health care system costs and treatment burdens associated with referral to a center capable of performing proton SRS (PSRS). Thus, understanding the practical strengths of each modality can inform shared decision-making between physicians and patients. Future direct PSRS vs photon SRS (XSRS) comparison clinical trials are unlikely. Dosimetric data from centers such as ours with extensive PSRS experience may elucidate potential benefits of PSRS and XSRS in representative clinical scenarios. In the present study, we plan and dosimetrically evaluate 9 representative cases of vestibular schwannoma using PSRS vs linac-based XSRS with 2 common multileaf collimator sizes.
Methods
Study Population
We identified a representative sample of patients with vestibular schwannomas among patients who received single-fraction PSRS between 2015 and 2018. The selected 9 cases varied in pertinent anatomic characteristics, including canal involvement, abutment/ proximity to the brainstem as assessed per Koos grade (Table 1, Figure 1), and clinical characteristics (Table 2). The study was approved by our institutional review board.
Simulation
Patients were immobilized with a modified Gill-Thomas-Cosman head frame (Integra-Radionics) and 1/16-inch diameter stainless steel fiducial markers were placed in the skull’s outer table to facilitate target volume alignment to the isocenter of the radiosurgical system.10,11 CT simulation with intravenous contrast was performed. Simulation images were obtained at 1.25-mm axial intervals and fused with diagnostic MRI to assist target delineation.
Treatment planning
PSRS plans were generated using the XiO planning system (Elekta Inc.). PSRS was delivered with a 3-D conformal, passive, single-scattering proton therapy unit via 3 equally or unequally weighted isocentric fields. Per department protocol, we defined case-specific lateral margins for penumbra and set-up uncertainty and a beam-specific, end-range margin with a 3.5% CT density correction plus 1 mm for range uncertainty.12
XSRS plans with volumetric-modulated arc therapy (VMAT) were generated using RayStation (RaySearch Laboratories) with 2.5-mm (2.5 XSRS) and 5-mm (5 XSRS) multileaf collimators (MLC) on the Varian Edge and TrueBeam systems, respectively, with a 6-MV flattening filter-free beam. VMAT plans were optimized with up to 3 partial arcs and avoided direct irradiation or exit dose through the ocular globes.
All cases were prescribed 12 Gy relative biological effectiveness (RBE) with standard RBE of 1.1 for protons. The gross target volume (GTV) encompassed radiographically apparent gross tumor. The planning target volume (PTV) consisted of the GTV with a 0.5- to 1.0-mm isotropic expansion. Dose heterogeneity was limited by ensuring an effective normalization of approximately 90%, while ensuring 98.8% PTV prescription coverage. Organs at risk (OAR) including brainstem, chiasm, cochlea, vestibule, ocular globes, hypothalamus, optic nerves, temporal lobe and brain, were verified by a neuro-anatomist and CNS-specialized radiation oncologist. For bilateral structures, the ipsilateral and contralateral volumes were delineated separately.
Plan Comparison
All plans were transferred to MIM software solutions for centralized, unbiased dosimetric comparison based on target volume dosimetry and estimated clinical toxicity.
Parameters assessed for target volume dosimetry included: 1) GTV coverage defined as the percent of the GTV receiving at least a given percentage of the prescription dose (V90%, V95% and V100%); 2) Dmax%, maximum percent dose to GTV defined as the highest percent of prescription dose to a 0.1 cc volume within the GTV; 3) homogeneity index, the maximum dose within the GTV divided by the prescription dose; 4) conformity index,13,14 the reference isodose volume divided by PTV target volume; and 5) gradient index (GI),15 defined as 50% of the prescription isodose volume divided by the prescription volume.
Clinical toxicity was estimated based on dose to OARs and excess risk of radiation-associated secondary intracranial tumor. The maximum dose to OARs (Dmax) was defined as the highest dose delivered to a 0.1 cc volume within the OAR with a 0.2 Gy buffer. To characterize inhomogeneous dose to each OAR, the equivalent uniform dose (EUD) was calculated. As first described by Niemierko, the EUD is the dose that when uniformly distributed over a given volume causes the same radiobiologic effect as the delivered nonuniform dose distribution.16 The computation is as follows , where where Di is the dose and vi is the partial volume of the i’th bin of the corresponding differential dose-volume histogram (DVH), and a is the model parameter specific to the OAR of interest.16 Parameter α was set to the following values: whole brain, 10; brainstem, 12; temporal lobes, 10; cochlea, 20; vestibule, 20; optic chiasm/ nerves/ocular globes, 10; and hypothalamus, 5.17
Excess risk of radiation-associated secondary intracranial tumor was modeled using the method proposed by Schneider based on organ equivalent dose, the dose that when uniformly distributed over a given volume causes the same radiation-induced tumor incidence as the delivered inhomogeneous dose.18 In the present study, organ equivalent dose is calculated using the dose-volume histogram for whole brain as , where the sum is taken over N bins of a differential DVH, vi is the relative size of the i’th bin corresponding to dose Di, and α is an organ-specific cell sterilization parameter. The excess risk of tumors (‘I’) is an organ-specific tumor incidence rate for a low radiation dose (I0) multiplied by the OED,
with the assumption that secondary tumor incidence rate is proportional to the number of mutated cells relative to the number of stem cells prior to irradiation. For intracranial irradiation, we use model parameters estimated by Schneider based on data published by the United Nation Scientific Committee on the Effects of Atomic Radiation (UNSCEAR) of I0 of 29.7 cancer cases per 10,000 patients per year per Sv and α = 0.08.
Parameters for target volume dosimetry and estimated clinical toxicity were evaluated using the Wilcoxon matched-pairs signed-ranks test and paired t-test with P value ≤ 0.05 considered statistically significant.
Results
The selected 9 cases of vestibular schwannoma represent diverse patient characteristics, tumor volumes and anatomic characteristics (Table 2). One patient underwent prior resection. Four lesions were either adjacent to or abutting the brainstem (Figure 1). The average target volume was 0.71 cc (range, 0.2-1.8). All cases were treated to a dose of 12 Gy (RBE).
Treatment plans were compared based on target volume dosimetry and estimated clinical toxicity. Figure 2 illustrates the differential dose distribution for each treatment modality with representative cross-sectional planning images for case 4, a patient with right-sided 0.6-cc vestibular schwannoma that abuts the brainstem. Summary target volume dosimetry metrics are shown in Table 3 (mean for all 9 cases according to modality and corresponding statistical comparisons). There were no significant differences in V100%, homogeneity index or Dmax% between treatment modalities. However, 5 XSRS and 2.5 XSRS offered equal or superior V90% and V95% compared with PSRS for all 9 cases. The gradient index, driven by the clinical directive of 98.8% PTV coverage, was highest for 5 XSRS (PSRS, 4.87; 5 XSRS, 5.98; 2.5 XSRS, 4.78). The conformity index was lowest for 2.5 XSRS (1.44) vs PSRS (1.59, P = NS) and 5 XSRS (1.61, P = 0.003).
Table 4 shows the Dmax in Gy (RBE) to pertinent OAR receiving > 1Gy (RBE) averaged across the 9 cases according to modality and corresponding statistical comparisons. Dmax to the ocular globes (ipsilateral and contralateral), optic nerves (ipsilateral and contralateral), optic chiasm, contralateral cochlea, contralateral vestibule, contralateral temporal lobe and hypothalamus was < 1 Gy (RBE) for each modality. Dmax to the ipsilateral temporal lobe was significantly lower with 2.5 XSRS, 7.7 compared with PSRS, 9.5, P = 0.001 and 5 XSRS, 8.2, P = 0.03. Dmax to ipsilateral cochlea was also lowest for 2.5 XSRS, 9.6 vs PSRS, 10.9, P = < 0.001 and 5 XSRS, 10.6, P = 0.007. Similarly, Dmax to ipsilateral vestibule was lowest for 2.5 XSRS, 7.7 vs PSRS, 8.7, P = 0.02 and vs 5 XSRS, 8.5, P = 0.02. Dmax to brainstem was lowest with PSRS, 8.6 vs 5 XSRS, 9.2, P = 0.04, and 2.5 XSRS, 8.8, P = NS.
The EUD to pertinent OAR in Gy (RBE) according to treatment modality for each case is shown in Figure 3 and numerical values for EUD averaged over the 9 cases for all OARs are provided in Supplementary Table 1 (online with article, www.appliedradiationoncology.com). The mean EUD to the ocular globes (ipsilateral and contralateral), optic nerves (ipsilateral and contralateral), chiasm, contralateral temporal lobe, hypothalamus, contralateral vestibule, and contralateral cochlea was < 1 Gy (RBE). OAR with significantly lower EUD in Gy (RBE) with 2.5 XSRS vs paired PSRS or 5 XSRS plans, respectively, included the ipsilateral cochlea (9.3, 10.3, 10.1), ipsilateral vestibule (7.7, 8.5, 8.4) and ipsilateral temporal lobe (3.8, 4.8, 4.2), P < 0.05 for 2.5 XSRS vs PSRS and 2.5 XSRS vs 5 XSRS. Mean EUD in Gy (RBE) to the brainstem was significantly lower with 2.5 XSRS, 4.7, and PSRS, 4.6, vs 5 XSRS, 5.0, P =.021 for both comparisons.
The projected excess risk of secondary tumor for each case according to treatment modality is graphically displayed in Figure 4 and corresponding numerical values are shown in Supplementary Table 2 (online with article, www.appliedradiationoncology.com). The estimated incidence of radiation-induced secondary tumors in cases per 10,000 patient-years was lowest for PSRS, 2.8, vs 5 XSRS, 6.6 and 2.5 XSRS, 5.5, (P < 0.008 for PSRS vs 2.5 XSRS, PSRS vs 5 XSRS and 5 XSRS vs 2.5 XSRS).
Discussion
In the present study, we rigorously compared the dosimetric advantages and limitations of PSRS, 5 XSRS and 2.5 XSRS by identifying 9 cases treated with PSRS representing various clinical characteristics and re-planning them with both 2.5 XSRS and 5 XSRS using the original treatment dose of 12 Gy (RBE) in 1 fraction. Our results demonstrate that metrics of target volume coverage and homogeneity are similar between modalities. The gradient and conformity indices were most optimal (closest to 1.0) for 2.5 XSRS. Regarding OAR, the mean Dmax and EUD to the ipsilateral temporal lobe, ipsilateral vestibule and ipsilateral cochlea were lowest with 2.5 XSRS. The relatively limited low-dose bath with PSRS was reflected in a projected excess risk of secondary tumor that was significantly different between treatment modalities — highest for 5 XSRS followed by 2.5 XSRS and nearly halved for PSRS.
Our results show that in treating vestibular schwannoma, dosimetric advantages are similar between PSRS and XSRS, but depending on clinical scenarios and acceptable tradeoffs, a given treatment modality might be favored. For many patients, select small statistically significant dosimetric advantages may not constitute a clinically relevant margin. Our formal dosimetric comparison to elucidate the subtleties of representative scenarios enables clinicians to decide when protons may be appropriate. These findings are important as dosimetric comparisons between PSRS and XSRS are limited4 and a randomized controlled trial between PSRS and XSRS is unlikely considering the large number of participants and long follow-up required to detect differences in tumor control and treatment toxicity. Furthermore, an informed decision in this setting may lower the additional health care system costs and individual patient treatment burden associated with referral to a center capable of performing PSRS.
Tumor control rates for modern series of vestibular schwannoma treated with XSRS delivered via Gamma Knife, CyberKnife or linac with tumor margin doses of equivalent to 12 Gy (RBE) in 1 fraction are upwards of 90%.19 A single institution series of 221 patients receiving proton radiation therapy (PSRS or fractionated), with approximately 62% of patients receiving PSRS using a passive scattering system, showed a 5-year tumor control rate of 96%.20 In seminal studies, doses > 12.5 to 13 Gy (RBE) were associated with increased morbidity with regard to cranial nerve toxicity (CN V, CN VII) without substantial gains in tumor control, whereas dose < 10 Gy (RBE) trended towards lower tumor control supporting modern dose regimens that aim to mitigate treatment toxicity.6,7,21
The impact of SRS on hearing preservation is controversial as there are inherent patient selection biases and variable findings in the literature with some series reporting a long-term decline in hearing22 and others reporting rates of hearing loss similar to observation.23 To maintain < 25% risk of serviceable hearing loss defined as hearing that is useful with or without a hearing aid, Quantitative Analysis of Normal Tissue Effects in the Clinic (QUANTEC) recommends a single-fraction maximum dose to the cochlea < 12 to 14 Gy.24,25 Our dosimetric analysis of dose to cochlea with PSRS and XSRS shows dose to ipsilateral cochlea is different between PSRS and 5 XSRS vs 2.5 XSRS with a small but potentially impactful difference applicable in situations where it is challenging to meet cochlear dose constraints.
Brainstem injury during SRS is typically due to treatment of adjacent lesions, especially vestibular schwannomas. Data in this setting are limited; however, in one of the largest vestibular schwannoma SRS series including 149 patients by Foote et al, significant risk factors for cranial neuropathy included tumor to brainstem distance, prior surgical resection and Dmax to brainstem with neuropathy rates of 2% vs 24% corresponding to dose < 12.5 vs > 12.5 Gy (RBE).21 Based on analysis of this study and other series, a Dmax of 12.5 Gy (RBE) to the brainstem during single-fraction SRS is recommended to limit the risk of permanent cranial neuropathy (due to proximity of cranial nerves to the brainstem) or necrosis to < 5% for patients with acoustic tumors.25,26 Our representative sample included tumors with varying distance from the brainstem. Although our small sample size may limit the ability to detect statistically significant differences, in these paired plans PSRS offered a lower brainstem Dmax compared with 2.5 XSRS and 5 XSRS. This difference may be clinically relevant in situations where tumor is in proximity to the brainstem.
Temporal lobe and brain parenchymal toxicity are anticipated to be low with typical vestibular schwannoma dose prescriptions of approximately 12 Gy (RBE). The volume of brain receiving 12 Gy is significantly associated with development of symptomatic postradiosurgical imaging changes27 and symptomatic radiation necrosis.28 The QUANTEC analysis recommends limiting the V12 Gy to < 5 to10 cc corresponding to a < 20% risk of symptomatic necrosis in single-fraction SRS.25 In our study, the prescription dose is 12 Gy (RBE) and the mean Dmax to ipsilateral temporal lobes was ≤ 9.5 Gy (RBE) across all modalities.
Reports of secondary tumors and malignant transformation are rare, as anticipated, given the low probability of these events and long latency period. In a retrospective series of 440 patients with vestibular schwannoma, median follow-up of 12.5 years, treated with Gamma Knife SRS between 1991 and 2000, 1 patient (0.03%) developed malignant transformation.29 Pollock et al performed a retrospective review of 1837 patients receiving SRS between 1990 and 2009 for benign tumors or indications with median follow-up of 9 years; they reported no radiation-induced tumors in 11,264 patient-years of follow-up and a predicted 5-, 10-, and 15-year risk of malignant transformation of 0.5%, 0.8% and 2.4%, respectively.30 Among the reported cases of malignant transformation in a vestibular schwannoma, 41% were in patients with neurofibromatosis, and reported histologies included malignant peripheral nerve sheath tumor, triton tumor, rhabdomyosarcoma and sarcoma.31 Based on a literature review and analysis of 36 cases of SRS-induced neoplasms, Patel and Chiang estimate the overall risk of developing an SRS-induced neoplasm is approximately 0.04% at 15 years; notably more than half of the initially treated tumors in this analysis were vestibular schwannomas.32 This is anticipated to be an underestimate as only a fraction of cases of secondary tumors are likely to be submitted as reports and accepted for publication. Furthermore, they note that the mean latency to development of an SRS-induced neoplasm is 7.9 years (range, 0.7-19 years),32 a duration that is relatively shorter than those observed with fractionated radiation therapy (median latency 15.2 ± 8.7 years in the pituitary adenoma experience).33 Historical series with long-term follow-up in the setting of fractionated radiation for pituitary adenoma report cumulative risk of second brain tumors of 2.0% at 10 years and 2.4% at 20 years without plateau,34 underscoring that the risk of secondary tumors even with older techniques is low but still a tangible risk that should be mitigated through careful modality selection and treatment planning.
To our knowledge, the present study is the first dosimetric comparison of PSRS with modern linac-based XSRS techniques specifically for treatment of vestibular schwannoma. Our results differ from dosimetric studies published 20 years ago but are similar to contemporary studies for other skull base tumors. For example, in a dosimetric comparison study of proton (spot scanning or passive scattering) and photon (3D conformal, stereotactic arc therapy, intensity-modulated RT) for benign brain tumors including 5 acoustic neuromas in 2003, Bolsi et al concluded that proton techniques were shown to be superior to all photon approaches for the irradiation of small brain lesions with regard to target dose uniformity, conformity and sparing of OAR.35 In contrast, our results show that neither modality has empirically superior dosimetry, which likely reflects technological progress in treatment planning, target localization and treatment delivery in recent decades leading to gains in photon dosimetry relative to proton dosimetry. Our results are consistent with the modern literature comparing proton and photon radiation for intracranial and skull base lesions, although there are no dedicated comparisons for vestibular schwannoma. In the setting of hypofractionated treatment (2-5 fractions) of intracranial tumors > 3 cm delivered via multiple modalities of SRS including protons (double-scattering proton therapy and intensity-modulated proton therapy) and photons (Gamma Knife, CyberKnife, and coplanar- and noncoplanar-arc VMAT), Cao et al showed that PSRS consistently offered the lowest integral dose to normal brain and most optimal homogeneity index, but each modality had dosimetric advantages and limitations on a case-by-case basis.36 In the setting of pituitary adenoma, PSRS compared with XSRS offered similar target volume dosimetry and a lower risk of radiation-induced secondary tumors.37 In the setting of conventional fractionation, Arvold et al38 and Winkfield et al39 report that for benign meningioma (mean target volume ~27 cc) and pituitary adenoma (target volume 2.4 cc), respectively, proton radiation compared with photon radiation decreased the risk of RT-associated secondary tumors and offered optimal OAR sparing. In our analysis, differences in OAR dose were modest, which may reflect similar small target volume dosimetry (mean target volume 0.71 cc) between photon and proton approaches. PSRS did offer a consistently lower risk of secondary tumors. In addition, 2.5 XSRS offered consistently equal target coverage and optimal OAR sparing relative to 5 XSRS, supporting use of this MLC size when available for linac-based SRS systems.
This study has several important limitations. The 9 cases were selected to be representative of common clinical scenarios applicable to vestibular schwannoma SRS — various anatomic characteristics, presentations, and prior resection and target volumes. However, this selection is not exhaustive. Many systems can deliver XSRS, including the linac, CyberKnife and Gamma Knife, among others. We focused our analysis on linac-based SRS, as this is a widely available modality in academic and community-based practices, and evaluated 2 common MLC sizes to address an important question in XSRS treatment planning. Our PSRS treatment planning is performed for a unique proton passive scattering system with optimized characteristics intended for small field delivery. Thus, our dosimetric assessments may not transfer to all other passive scattering systems and may not directly apply to pencil-beam scanning with or without aperture collimation. SRS plans can be modified based on planning priorities and resources. The plans presented in our study may differ from those generated at other institutions. As described in our methods, we used commercially available software and common planning criteria; thus, major variations from our data can occur but would be unlikely.
Conclusion
In conclusion, this study compared the dosimetric advantages and limitations of PSRS, 5 XSRS and 2.5 XSRS for vestibular schwannoma. We show similar target coverage and OAR sparing with XSRS and PSRS; 2.5 XSRS offers greater target conformality and lower dose to OAR than 5 XSRS, and PSRS offers significantly lower excess risk of secondary tumor than XSRS, although the absolute risk of secondary tumors is low across modalities.
References
- Carlson ML, Link MJ. Vestibular schwannomas. Ingelfinger JR, ed. N Engl J Med. 2021;384(14):1335-1348. doi:10.1056/NEJMra2020394
- Reznitsky M, Petersen MMBS, West N, Stangerup SE, Cayé-Thomasen P. Epidemiology of vestibular schwannomas – prospective 40-year data from an unselected national cohort. Clin Epidemiol. 2019;(11):981-986. doi:10.2147/CLEP.S218670
- Marinelli JP, Lohse CM, Carlson ML. Incidence of vestibular schwannoma over the past half-century: a population-based study of Olmsted County, Minnesota. Otolaryngol Neck Surg. 2018;159(4):717-723. doi:10.1177/0194599818770629
- Olson JJ, Kalkanis SN, Ryken TC. Congress of Neurological Surgeons systematic review and evidence-based guidelines on the treatment of adults with vestibular schwannomas: executive summary. Neurosurgery. 2018;82(2):129-134. doi:10.1093/neuros/nyx586
- Murphy ES, Suh JH. Radiotherapy for vestibular schwannomas: a critical review. Int J Radiat Oncol. 2011;79(4):985-997. doi:10.1016/j.ijrobp.2010.10.010
- Combs SE, Welzel T, Schulz-Ertner D, Huber PE, Debus J. Differences in clinical results after LINAC-based single-dose radiosurgery versus fractionated stereotactic radiotherapy for patients with vestibular schwannomas. Int J Radiat Oncol Biol Phys. 2010;76(1):193-200. doi:10.1016/j.ijrobp.2009.01.064
- Mendenhall WM, Friedman WA, Buatti JM, Bova FJ. Preliminary results of linear accelerator radiosurgery for acoustic schwannomas. J Neurosurg. 1996;85(6):1013-1019. doi:10.3171/jns.1996.85.6.1013
- Koetsier KS, Hensen EF, Wiggenraad R, et al. Clinical outcomes and toxicity of proton radiotherapy for vestibular schwannomas: a systematic review. J Radiat Oncol. 2019;8(4):357-368. doi:10.1007/s13566-019-00410-1
- Persson O, Bartek J, Shalom NB, Wangerid T, Jakola AS, Förander P. Stereotactic radiosurgery vs. fractionated radiotherapy for tumor control in vestibular schwannoma patients: a systematic review. Acta Neurochir (Wien). 2017;159(6):1013-1021. doi:10.1007/s00701-017-3164-6
- Gall KP, Verhey LJ, Wagner M. Computer-assisted positioning of radiotherapy patients using implanted radiopaque fiducials. Med Phys. 1993;20(4):1153-1159. doi:10.1118/1.596969
- Winey B, Daartz J, Dankers F, Bussière M. Immobilization precision of a modified GTC frame. J Appl Clin Med Phys. 2012;13(3):3690. doi:10.1120/ jacmp.v13i3.3690
- Paganetti H. Range uncertainties in proton therapy and the role of Monte Carlo simulations. Phys Med Biol. 2012;57(11):R99-R117. doi:10.1088/0031-9155/57/11/R99
- Shaw E, Kline R, Gillin M, et al. Radiation therapy oncology group: radiosurgery quality assurance guidelines. Int J Radiat Oncol. 1993;27(5):1231-1239. doi:10.1016/0360-3016(93)90548-A
- Feuvret L, Noël G, Mazeron JJ, Bey P. Conformity index: a review. Int J Radiat Oncol. 2006;64(2):333-342. doi:10.1016/j.ijrobp.2005.09.028
- Paddick I, Lippitz B. A simple dose gradient measurement tool to complement the conformity index. J Neurosurg. 2006;105 Suppl:194-201. doi:10.3171/ sup.2006.105.7.194
- Niemierko A. Reporting and analyzing dose distributions: a concept of equivalent uniform dose. Med Phys. 1997;24(1):103-110. doi:10.1118/1.598063
- Gay HA, Niemierko A. A free program for calculating EUD-based NTCP and TCP in external beam radiotherapy. Phys Med. 2007;23(3-4):115-125. doi:10.1016/j. ejmp.2007.07.001
- Schneider U, Zwahlen D, Ross D, Kaser-Hotz B. Estimation of radiation-induced cancer from three-dimensional dose distributions: concept of organ equivalent dose. Int J Radiat Oncol. 2005;61(5):1510-1515. doi:10.1016/j.ijrobp.2004.12.040
- Soltys SG, Milano MT, Xue J, et al. Stereotactic radiosurgery for vestibular schwannomas: tumor control probability analyses and recommended reporting standards. Int J Radiat Oncol. 2021;110(1):100-111. doi:10.1016/j.ijrobp.2020.11.019
- Koetsier KS, Hensen EF, Niemierko A, et al. Outcome and toxicity of proton therapy for vestibular schwannoma: a cohort study. Otol Neurotol Off Publ Am Otol Soc Am Neurotol Soc Eur Acad Otol Neurotol. 2021;42(10):1560-1571. doi:10.1097/MAO.0000000000003313
- Foote KD, Friedman WA, Buatti JM, Meeks SL, Bova FJ, Kubilis PS. Analysis of risk factors associated with radiosurgery for vestibular schwannoma. J Neurosurg. 2001;95(3):440-449. doi:10.3171/jns.2001.95.3.0440
- Carlson ML, Jacob JT, Pollock BE, et al. Long-term hearing outcomes following stereotactic radiosurgery for vestibular schwannoma: patterns of hearing loss and variables influencing audiometric decline. J Neurosurg. 2013;118(3):579-587. doi:10.3171/2012.9.JNS12919
- Breivik CN, Nilsen RM, Myrseth E, et al. Conservative management or gamma knife radiosurgery for vestibular schwannoma: tumor growth, symptoms, and quality of life. Neurosurgery. 2013;73(1):48-56; discussion 56-57. doi:10.1227/01.neu.0000429862.50018.b9
- Bhandare N, Jackson A, Eisbruch A, et al. Radiation therapy and hearing loss. Int J Radiat Oncol. 2010;76(3):S50-S57. doi:10.1016/j.ijrobp.2009.04.096
- Marks LB, Yorke ED, Jackson A, et al. Use of normal tissue complication probability models in the clinic. Int J Radiat Oncol. 2010;76(3):S10-S19. doi:10.1016/j. ijrobp.2009.07.1754
- Mayo C, Yorke E, Merchant TE. Radiation associated brainstem injury. Int J Radiat Oncol. 2010;76(3):S36-S41. doi:10.1016/j.ijrobp.2009.08.078
- Flickinger JC, Kondziolka D, Pollock BE, Maitz AH, Lunsford LD. Complications from arteriovenous malformation radiosurgery: multivariate analysis and risk modeling. Int J Radiat Oncol Biol Phys. 1997;38(3):485-490. doi:10.1016/s0360-3016(97)89481-3
- Korytko T, Radivoyevitch T, Colussi V, et al. 12 Gy Gamma Knife radiosurgical volume is a predictor for radiation necrosis in non-AVM intracranial tumors. Int J Radiat Oncol Biol Phys. 2006;64(2):419-424. doi:10.1016/j.ijrobp.2005.07.980
- Hasegawa T, Kida Y, Kato T, Iizuka H, Kuramitsu S, Yamamoto T. Long-term safety and efficacy of stereotactic radiosurgery for vestibular schwannomas: evaluation of 440 patients more than 10 years after treatment with Gamma Knife surgery. J Neurosurg. 2013;118(3):557-565. doi:10.3171/2012.10.JNS12523
- Pollock BE, Link MJ, Stafford SL, Parney IF, Garces YI, Foote RL. The risk of radiation-induced tumors or malignant transformation after single-fraction intra-cranial radiosurgery: results based on a 25-year experience. Int J Radiat Oncol. 2017;97(5):919-923. doi:10.1016/j.ijrobp.2017.01.004
- Seferis C, Torrens M, Paraskevopoulou C, Psichidis G. Malignant transformation in vestibular schwannoma: report of a single case, literature search, and debate: case report. J Neurosurg. 2014;121(Suppl_2):160-166. doi:10.3171/2014.7.GKS141311
- Patel TR, Chiang VLS. Secondary neoplasms after stereotactic radiosurgery. World Neurosurg. 2014;81(3-4):594-599. doi:10.1016/j.wneu.2013.10.043
- Yamanaka R, Abe E, Sato T, Hayano A, Takashima Y. Secondary intracranial tumors following radiotherapy for pituitary adenomas: a systematic review. Cancers. 2017;9(12):103. doi:10.3390/cancers9080103
- Minniti G, Traish D, Ashley S, Gonsalves A, Brada M. Risk of second brain tumor after conservative surgery and radiotherapy for pituitary adenoma: update after an additional 10 years. J Clin Endocrinol Metab. 2005;90(2):800-804. doi:10.1210/jc.2004-1152
- Bolsi A, Fogliata A, Cozzi L. Radiotherapy of small intracranial tumours with different advanced techniques using photon and proton beams: a treatment planning study. Radiother Oncol J Eur Soc Ther Radiol Oncol. 2003;68(1):1-14. doi:10.1016/s0167-8140(03)00117-8
- Cao H, Xiao Z, Zhang Y, et al. Dosimetric comparisons of different hypofractionated stereotactic radiotherapy techniques in treating intracranial tumors > 3 cm in longest diameter. J Neurosurg. 2020;132(4):1024-1032. doi:10.3171/2018.12.JNS181578
- Sud S, Botticello T, Niemierko A, Daly J, Bussiere M, Shih HA. Dosimetric comparison of proton versus photon radiosurgery for treatment of pituitary ad- enoma. Adv Radiat Oncol. 2021;6(6):100806. doi:10.1016/j.adro.2021.100806
- Arvold ND, Niemierko A, Broussard GP, et al. Projected second tumor risk and dose to neurocognitive structures after proton versus photon radiotherapy for benign meningioma. Int J Radiat Oncol. 2012;83(4):e495-e500. doi:10.1016/j.ijrobp.2011.10.056
- Winkfield KM, Niemierko A, Bussière MR, et al. Modeling intracranial second tumor risk and estimates of clinical toxicity with various radiation therapy techniques for patients with pituitary adenoma. Technol Cancer Res Treat. 2011;10(3):243-251. doi:10.7785/tcrt.2012.500199
Citation
Sud S, Bussiere M, Botticello T, Niemierko A, Schwartz A, Shih HA. Dosimetric Comparison of Proton Versus Photon Stereotactic Radiosurgery for Treatment of Vestibular Schwannoma. Appl Radiat Oncol. 2023;(1):20-30.
March 21, 2023